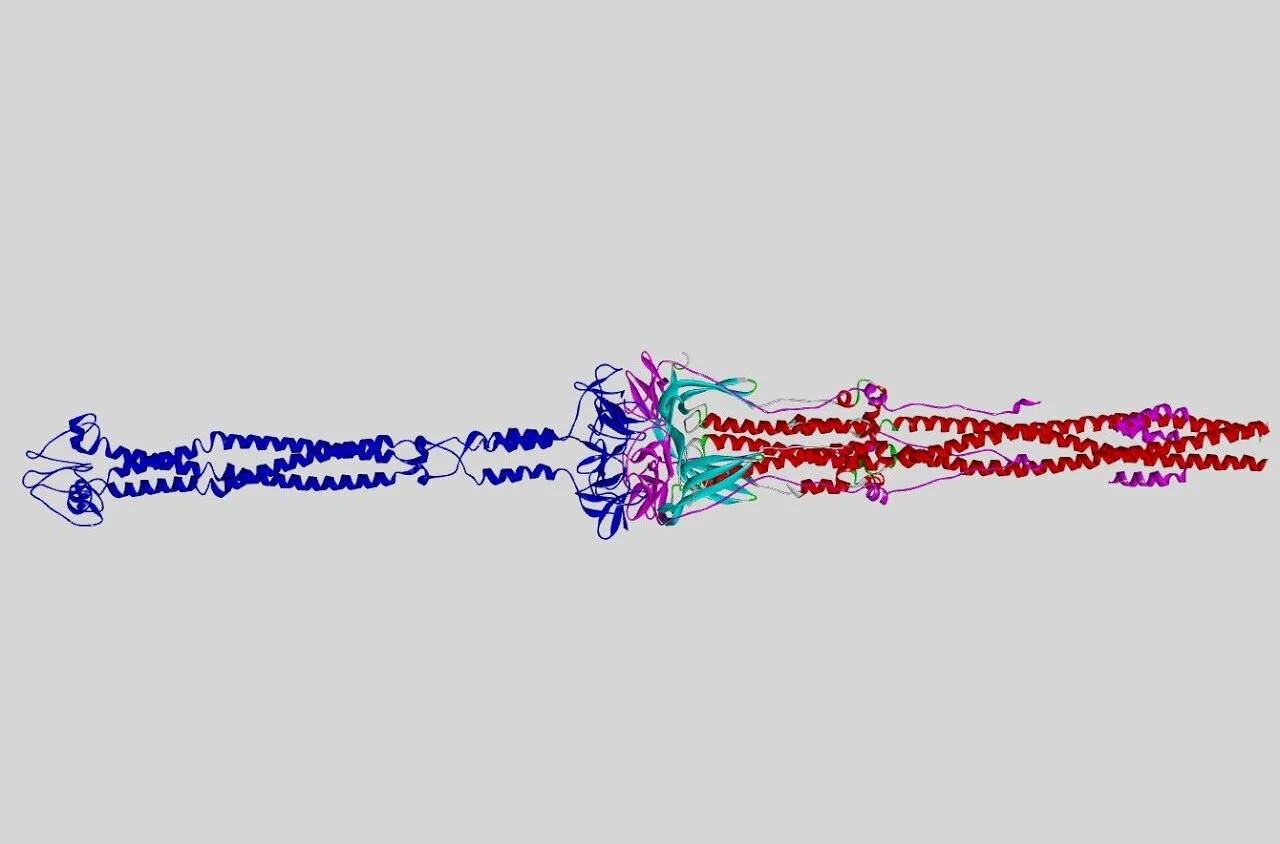
Modelling the Viriron's Components.
The bircks and morter of the macro-model.
Covid-19 Home Introduction Background Method Downloads Models
The Method Behind the Modelling
Membrane
The major component of the SARS-CoV-2 virion is the virus envelope, this is mostly composed of phospholipid molecules arranged to form a bilayer (1). It has been shown some envelope viruses can alter the hosts lipidome profile towards their advantage (2), so presuming the envelope is composed of normal proportions of plasma membrane lipids, is not necessarily correct. As coronaviruses bud in the intermediate compartment at the endoplasmic reticulum Golgi interface the viral envelope is composed of lipids found at these cellular organelles. The exact lipids and their proportions are difficult to determine. Lipid molecule species vary from one organelle to another, the proportions and even type found in the Golgi Apparatus can be distinct from those found at cell membrane (3).
SEM and TEM images of SARS-CoV-2 viral fusion at the plasma membrane, taken from (Djamal, BB, 2020).
The Endoplasmic Reticulum (ER) and Golgi membrane has higher levels of Phosphatidylcholine (PtdCho) and Phosphatidylethanolamine (PtdEtn) phospholipids, while lower levels of cholesterol compared to the plasma membrane. One study on rat liver cells reports molecular lipid percentages for the ER of PtdCho 58%, PtdEtn 22%, Phosphatidylserine (PtdSer) 3%, Phosphatidylinositol (PtdIns) 10%, Sphingomyelin (Sm) 3%, and Cholesterol 0.08%, while the Golgi membrane presents proportions of PtdCho 50%, PtdEtn 20%, PtdSer 6%, PtdIns 12%, Sm 8%, and Cholesterol 0.16% (4).
The Alphafold model of the Membrane (M) protein did take account of membrane molecular dynamics in determining the protein structure. We chose to use the same proportions of lipid types used by the AlphaFold team; 55% POPC, 25% POPE, 10% POPI, 2% POPS, 6% Cholestreol, and 2% cardiolipin (5).
Top: PtdCho 1-palmitoyl-2-oleoyl-sn-glycero-3-phosphocholine (POPC)
Bottom: PtdSer like 1-palmitoyl-2-oleoyl-sn-glycero-3-phospho-l-serine (POPS)
Top: PtdEtn 1-Palmitoyl-2-oleoyl-sn-glycero-3-phosphoethanolamine (POPE)
Bottom: Cholesteral
Top: PtdIns Phosphatidylinositol (POPI) .
Bottom: Cardiolipin
We used the online in silico MemGen tool (6) to produce a lipid bilayer tile, this measured 70 by 70 angstroms populated with the established lipid molecules in their associated proportions. This was rendered as a PDB file for editing.
Proteins
Of the four structural proteins, the Spike (S), Membrane and Envelope (E) proteins are present and “visible” on the outside of the virion. The nucleocapsid (N) protein is responsible for the packaging of the virus’ RNA code and only present on the inside of the envelope (7). At this stage of our modelling, we have chosen not to include the N proteins or the RNA in our macromolecular structure. This partly because of time and effort constraints. Also, a clear consensus for the internal structural microbiology of the virion is still emerging (8).
Additionally, several of the viral proteins are heavily glycosylated, paticularly the S protein (9). Glycosylation is the process of adding monosaccharides (sugars) to the backbone of proteins (10). Viruses can use these glycans hijacked from the host’s cellular machinery to hide viral proteins from the cells immune response (11). Maps of N-linked and O-linked glycosylation on the S protein are already well documented, but given glycan groups are highly mobile, modelling them effectively on a static structure has its paticularly difficulties (11). We have chosen to show the SARS-CoV-2 proteins without any posttranslational modifications including glycosylation. We hope to have the opportunity to update the model in the future to include the N protein, RNA, and glycosylation groups.
Charmm-GUI 6VSB transmembrane domain model.
On the virion each spike protein structure presents as a trimer (18) – that is three peptide chains of the same sequence are required to form one functional Spike protein. The S protein can adopt numerous conformations or shapes. In our model we chose to include four key conformations. The closed conformation presents all three RBDs in a down position, in this state the Spike protein cannot interact with the ACE2 entry receptor (18) – it is possible this conformation acts to assist in the construction of the virion, preserving the Spike protein during component assembly much like a flick-knife protects the blade from unwanted use (19).
Top: Spike protein in the open conformation.
Bottom: Spike protein unit in the double-open conformation.
Finally, the post-fusion state is the most radically different Spike structure. This form of the S protein has lost the associated S1 regions including the RBDs, the post fusion Spike protein consists of only the S2 and TM domains rendering it non-infectious (22). In the fusion process the cleavage of the S1 region is thought to be assisted by the host TMPRSS2 enzyme and is a mechanical part of viral-cell fusion (23). However, the loss of the S1 region is thought to occasionally occur spontaneously (22). Post-fusion Spike proteins are thought to occur on mature viable virions in small but notable quantities. These may help the virus evade antibody recognition or maintain structural rigidity (22).
The Spike protein
Multiple structures for the Spike protein have been determined using cryo-electron microscopy. These have resolved both the S1 region, which possess the receptor binding domain (RBD) responsible for ACE2 recognition, and the S2 region responsible for membrane fusion (12-13). The transmembrane (TM) region of the S protein has not been directly resolved due to difficulties in imagining membrane bound proteins (14). However, teams at both Charmm and Zhang Lab have produced full models of the S protein which include the transmembrane region (15-16). We based our TM complete membrane bound spike protein models on the structured produced using Charmm (17).
The Spike protein trimer unit in the closed conformation.
The open conformation presents one RBD in an erect position ready to interact with the entry receptor ACE2, while the other two RBDs are lowered. This appears to be the most common state of Spike proteins on mature prefusion virions (20).
The double open conformation has two RBDs in an erect state while one in a closed state. This is a relatively rare conformation, perhaps because although it may promote ACE2 receptor interactions, it could also expose the virus to a stronger antigen response from the host’s immune system (21).
Post-fusion spike protein.
We used a structural overlay including several S protein models for each conformation, for example 6VYB, 6CRV, 6XR8, (18,22,24) but primary the PDB structures used for the model were 6VXX for the closed state, 6VSB for the open state, 6X2B for the double open state and 6XRA for the post-fusion state (18-22). The Charmm models for 6VXX and 6VSB had proposed transmembrane regions (17).
Adjoining the TM region to the 6X2B structure was relatively simple, the process was more complex for the post-fusion model 6XRA. We inspected and overlayed the quite distinct open conformation 6VSB with the post-fusion 6XRA structure. A stretch of residues from R1000 to F1075 which lies at the core of the structure between the heptad repeat 1 (HR1) and HR2 domains, towards the C end of the peptide chain, (25) were of paticular importance. This region showing a close structural similarity across the pre- and post-fusion structures.
The conserved structural overlay of the pre-fusion (standard colouring) and post-fusion (green) states of the S protein units. the residues shown are found between R1000 to F1075.
Our proposed model for the transmembrane-included post-fusion S protein. Transmembrane region in blue, included post-fusion structure in standard colouring and the proposed incorrectly determined flexable loop in pink, which was removed.
The current stand model of the transmembrane location of the post-fusion S protein figure taken from Cai, Y et al. (22).
This region was used to orientate the transmembrane end of the post-fusion structure, placing the transmembrane domain proximal to N703 of the resolved structure. Although in the post-fusion structure the C-end of the peptide chain doubles back on the structure ending near the N terminal. We hypothesis this could be a none-native conformation, the C-end acting as a flexible loop. We understand this is not the consensus view among the literature. Models of the transmembrane-complete post fusion Spike protein render it as a club like shape, with the transmembrane region adjoined the opposite end proximal to T912 (22). We replace the post-fusion structure from F1075 onwards with the transmembrane region from 6VSB so orientating the peptide chain with the N terminal at the furthest point from the virus envelope and the C terminal ending on the inside of the virus - so forming a lance like model.
M protein
As discussed previously, the M protein has not been directly resolved, instead ab initio models have been proposed. We chose to include Alphafold’s prediction of the M protein structure (26), this based on the programs previous success at folding predictions, the fact the structure was a well packed dimmer and the use of ORF3a in both confirming the initial structure and refining the updated model (27-28).
Additionally, electron micrographs focusing on the M protein show it to be wedge-like in shape with the thick-end protruding into the envelope’s interior and the thin-end barely rising above the external side of the virion’s lipid bilayer (29). This is in-keeping with the proposed function of the M protein where its interactions with the Spike and N proteins help to keep the constituent parts secured and whole with the virion’s lipid envelope (30). Other models of the M protein propose shorter loops protruding from the either side of the bilayer and do not show as clearer symmetry for dimerization (31).
The 7K3G pdb structure each of the 5 subunits coloured differently.
The Alphafold prediction for the dimeric M protein strucure, one unit in standard colouring, the other in blue.
E protein
Until recently only homology models existed for the E protein (31). However, in late 2020 the structure was resolved using NMR techniques (PDB 7K3G)(32), we used this model in our macro-structure. The E protein is composed of 5 small identical peptides these span the lipid bilayer as alpha helices arrange in a five-helix bundle. It is thought the structure acts as an ion-channel and primarily plays a role in viral budding (32). E proteins occurring in small numbers on the mature virion but whether they continue to fulfil a function after budding is unclear.
The distrubution of hydrophobicity across the M protein's surface, (blue indicting charged regions).
The distrubution of hydrophobicity across the E protein's surface.
The distrubution of hydrophobicity across the transmembrane region of the S protein's surface.
Setting the proteins
The transmembrane regions of the Spike proteins, M protein, and E protein were inspected for their surface hydrophobicity and were set into our 70 angstrom2 lipid bilayer model. We found the E protein exhibited strong hydrophobicity along it’s side, the top and bottom were more charged and could not protrude above the charged layers of the phospholipids, instead being buried among the envelope. The M protein was placed with the thick wedge end inset to the charge layer of the phospholipids in accordance with hydrophobicity surface render. Finally, the transmembrane domain of the spike proteins were set similarly to the M protein, the most hydrophobic region placed where the bilayer meets.
References:
Schoeman, D, Fielding, BC 2019, ‘Coronavirus envelope protein: current knowledge’. Virol. J., vol. 16, no. 69, https://doi.org/10.1186/s12985-019-1182-0.
Abu-Farha, M, Thanaraj, TA, Qaddoumi, MG, Hashem, A, Abubaker, J, Al-Mulla, F 2020, ‘The Role of Lipid Metabolism in COVID-19 Virus Infection and as a Drug Target’. Int. J. Mol. Sci., vol. 21, no. 10, pp. 3544, doi:10.3390/ijms21103544.
Casares, D, Escribá, PV, Rosselló, CA 2019, ‘Membrane Lipid Composition: Effect on Membrane and Organelle Structure, Function and Compartmentalization and Therapeutic Avenues’. Int. J. Mol. Sci. vol. 20, no. 9, pp. 2167, doi:10.3390/ijms20092167.
Meer GV 1998, ‘Lipids of the Golgi membrane’, trends in Cell Biology, vol. 8.
Heo L and AlphaFold colleagues, SARS-Cov-2 protein structure models, GitHub, viewed 21 April 2021, <https://github.com/feiglab/sars-cov-2-proteins>.
Knight, CJ, Hub JS 2015, ‘MemGen: A general web server for the setup of lipid membrane simulation systems’. Bioinformatics, vol. 31, pp. 2897-2899, doi:10.1093/bioinformatics/btv292.
Kang, S, Yang, M, Hong, Z, Zhang, L 2020 ‘Crystal structure of SARS-CoV-2 nucleocapsid protein RNA binding domain reveals potential unique drug targeting sites’, Acta. Pharmaceutica Sinica. B., vol. 10, no. 7, pp. 1228-1238, https://doi.org/10.1016/j.apsb.2020.04.009.
Masters, PS 2019, ‘Coronavirus genomic RNA packaging’, Virology, vol. 537, pp. 198-207, https://doi.org/10.1016/j.virol.2019.08.031.
Liangwei, D, Qianqian, Z, Hongxia, Z, Yuna, N, Yunwei, L, Hui W 2020, ‘The SARS-CoV-2 Spike Glycoprotein Biosynthesis, Structure, Function, and Antigenicity: Implications for the Design of Spike-Based Vaccine Immunogens.”, Frontiers in Immunology, vol. 11, pp. 2593, https://www.frontiersin.org/article/10.3389/fimmu.2020.576622.
SimGlycan 2021, Protein Glycosylation, Premier Biosoft, viewed 21 April 2021, <http://www.premierbiosoft.com/glycan/glossary/glycosylation.html>.
Grant, OC, Montgomery, D, Ito, K, et al. 2020, ‘Analysis of the SARS-CoV-2 spike protein glycan shield reveals implications for immune recognition.’ Sci. Rep., vol 10, no. 14991, https://doi.org/10.1038/s41598-020-71748-7.
Ou, X, Liu, Y, Lei, X, et al. 2020, ‘Characterization of spike glycoprotein of SARS-CoV-2 on virus entry and its immune cross-reactivity with SARS-CoV.’, Nat. Commun., vol. 11, no. 1620, https://doi.org/10.1038/s41467-020-15562-9.
Huang, Y, Yang, C, Xu, XF, et al. 2020, ‘Structural and functional properties of SARS-CoV-2 spike protein: potential antivirus drug development for COVID-19.’ Acta. Pharmacol. Sin., vol. 41, pp. 1141–1149, https://doi.org/10.1038/s41401-020-0485-4.
Choi YK, Cao Y, Frank M, Woo H, et al. 2021, ‘Structure, Dynamics, Receptor Binding, and Antibody Binding of the Fully Glycosylated Full-Length SARS-CoV-2 Spike Protein in a Viral Membrane.’, J. Chem. Theory Comput., vol. 17, no. 4, pp. 2479-2487, doi: 10.1021/acs.jctc.0c01144.
Woo, H, Park, SJ, Choi, YK, Park, T, et al. 2020, ‘Developing a Fully Glycosylated Full-Length SARS-CoV-2 Spike Protein Model in a Viral Membrane.’, The Journal of Physical Chemistry B, vol. 124, no. 33, pp. 7128-7137, DOI: 10.1021/acs.jpcb.0c04553.
Zhang Lab 2020, Covid-19, University of Michigan, viewed 23 April 2021, <https://zhanglab.ccmb.med.umich.edu/COVID-19/>.
Charmm-GUI 2020, CHARMM-GUI Archive - COVID-19 Proteins Library, Lehigh University, viewed 21 April 2021, <https://charmm-gui.org/?doc=archive&lib=covid19>.
Walls, AC, Park, JY, Tortorici, MA, Wall, A, et al. 2020, ‘Structure, Function, and Antigenicity of the SARS-CoV-2 Spike Glycoprotein.’, Cell, vol. 181, no. 2, pp. 281-292.e6, https://doi.org/10.1016/j.cell.2020.02.058.
Qu, K, Xiong, X, Ciazynska, KA, Carter, AP, Briggs, JAG 2021, ‘Structures and function of locked conformations of SARS-CoV-2 spike.’, [preprint], bioRxiv 2021.03.10.434733; doi: https://doi.org/10.1101/2021.03.10.434733.
Wrapp, D, Wang, N, Corbett, KS, Goldsmith, JA et al. 2020, ‘Cryo-EM structure of the 2019-nCoV spike in the prefusion conformation.’, Science, pp. 1260-1263.
Henderson, R, Edwards, RJ, Mansouri, K, et al. 2020, ‘Controlling the SARS-CoV-2 spike glycoprotein conformation.’, Nat. Struct. Mol. Biol. vol. 27, pp. 925–933, https://doi.org/10.1038/s41594-020-0479-4.
Cai, Y, Zhang, J, Xiao, T, Peng, H, et al. 2020, ‘Distinct conformational states of SARS-CoV-2 spike protein.’, Science, pp. 1586-1592.
Hoffmann, M, Kleine-Weber, H, Schroeder, S, Krüger, N 2020 ‘SARS-CoV-2 Cell Entry Depends on ACE2 and TMPRSS2 and Is Blocked by a Clinically Proven Protease Inhibitor’, Cell, vol. 181, no. 2, pp. 271-280, https://doi.org/10.1016/j.cell.2020.02.052.
Kirchdoerfer, RN, Wang, N, Pallesen, J et al. 2018, ‘Stabilized coronavirus spikes are resistant to conformational changes induced by receptor recognition or proteolysis.’, Sci. Rep., vol. 8, no. 15701, https://doi.org/10.1038/s41598-018-34171-7.
Xia, S, Liu, M, Wang, C et al. 2020, ‘Inhibition of SARS-CoV-2 (previously 2019-nCoV) infection by a highly potent pan-coronavirus fusion inhibitor targeting its spike protein that harbors a high capacity to mediate membrane fusion.’ Cell Res., vol. 30, pp. 343–355, https://doi.org/10.1038/s41422-020-0305-x.
Jumper, J et al. 2020, Computational predictions of protein structures associated with COVID-19, DeepMind website, viewed 21 April 2021, <https://deepmind.com/research/open-source/computational-predictions-of-protein-structures-associated-with-COVID-19>.
Callaway E 2020, ‘It will change everything’: DeepMind’s AI makes gigantic leap in solving protein structures, Nature [News], viewed 20 April 2021, <https://www.nature.com/articles/d41586-020-03348-4>.
Kern, DM, Sorum, B, Mali, SS, et al. 2020, ‘Cryo-EM structure of the SARS-CoV-2 3a ion channel in lipid nanodiscs’. [preprint] bioRxiv 2020.06.17.156554; doi: https://doi.org/10.1101/2020.06.17.156554.
Neuman, BM, Kiss, G, Kunding, AH, Bhella, D et al. 2011, ‘A structural analysis of M protein in coronavirus assembly and morphology.’, J. Struct. Biol., vol. 174, no. 1, pp. 11–22, doi: 10.1016/j.jsb.2010.11.021.
Siu, YL, Teoh, KT, Lo, J, Chan, CM, et al. 2008, ‘The M, E, and N Structural Proteins of the Severe Acute Respiratory Syndrome Coronavirus Are Required for Efficient Assembly, Trafficking, and Release of Virus-Like Particles.’, Journal of Virology, vol. 82, no. 22, pp. 11318-11330, DOI: 10.1128/JVI.01052-08.
Andrade, MA, Bianchi, M, Benvenuto, D, Giovanetti, M et al. 2020, ‘Sars-CoV-2 Envelope and Membrane Proteins: Structural Differences Linked to Virus Characteristics?’, BioMed Research International, vol. 2020, no. 4389089, https://doi.org/10.1155/2020/4389089.
Mandala, VS, McKay, MJ, Shcherbakov, AA et al. 2020, ‘Structure and drug binding of the SARS-CoV-2 envelope protein transmembrane domain in lipid bilayers.’, Nat. Struct. Mol. Biol., vol. 27, pp. 1202–1208, https://doi.org/10.1038/s41594-020-00536-8.
Photo credits: Djamal, BB, Anthony, F, Jean-Pierre, B, Gabriel, H. et al. 2020, ‘The Strengths of Scanning Electron Microscopy in Deciphering SARS-CoV-2 Infectious Cycle.’, Frontiers in Microbiology, vol. 11, pp. 2014.